Prompt
Enhanced rock weathering is a method to remove carbon dioxide from the atmosphere and store it permanently. Some of its best features are simplicity, permanence, and scale. The mechanism is that carbon dioxide in the air reacts with rock particles to form stable carbonates. There are many times more rocks than we need to remove industrial-era emissions.
"Why aren't we grinding up mountains yet?" is a question I've been curious about. Folks from Stripe Frontier and Orca Sciences have taught me a lot about the topic.
The promise is still there, but the road is more challenging than at first glance. The difficulty increases after considering rock quality, reaction kinetics, logistics, ocean impacts, mining operations, and MRV (Measurement, Reporting, and Verification).
Intro
We have more carbon dioxide in the atmosphere than we'd like, and it is an acid gas. Acid gases form acidic solutions when they dissolve in water. The magic of enhanced rock weathering is finding basic rocks that can react with and neutralize CO2 into bicarbonate or precipitated carbonates. Below are the reactions for olivine, which is one of the most commonly proposed rock types:
Mg2SiO4 + 4CO2 + 2H2O => 2Mg2+ + 4HCO3- + SiO2
Each ton of olivine removes 1.25 tons of CO2 in the aqueous reaction. This reaction is only relevant for dumping the rocks in the ocean because smaller reservoirs can't maintain that much bicarbonate.
There is also a route that forms carbonates:
Mg2SiO4 + 2CO2 => 2MgCO3 + SiO2
The reaction is two steps, with the first forming bicarbonate ions and the second reaction producing carbonates:
Mg2+ + 2(HCO3-) => MgCO3 + CO2 + H2O
The carbonate reaction does not store as much CO2 as the bicarbonate phase.
Overall, the CO2/bicarbonate/carbonate buffer system is integral to how rock weathering proceeds.
Evaluating Previous Cost Claims
Part of the hype around rock weathering is claims that it could be done for $10/ton of CO2 removed at scale. A cost like this would make a gallon of fuel carbon neutral for only ~$0.10.
Diving further into the costs suggests that the conditions where this might be true are strict:
-
Tens of millions of tons per year scale.
-
Extremely large, contiguous, and high purity reserves with minimal overburden.
-
Inexpensive electricity (<$20/MWh).
-
Zero cost of capital.
-
No verification, testing, or pollution concerns.
-
Ocean disposal to get the bicarbonate bonus.
-
A mine located less than 5 kilometers from the ocean.
-
The ocean is the warm water variety to speed reaction rates.
-
Acceptance of long time horizons or new grinding technology.
It isn't clear whether these conditions are available in any location on Earth. High-quality reserves tend to be in smaller veins within larger deposits. Existing olivine mines are small and far from the ocean or in cold places. Pollution is a concern because deposits usually contain small amounts of metals like nickel that can cause localized issues in the ocean. The material might not fully react before settling on the bottom, and measuring this is challenging.
Quotes for high-purity olivine from existing mines are over $100/ton because of their sub-scale nature and the mining difficulty. One of the main mines in the US, Twin Sisters in Washington, isn't even accessible year-round.
$10/ton does not seem realistic.
Land-Based Weathering
Nan, Dan, and Judy at Frontier suggested that land-based weathering might be under-explored because transportation, pollution, land use, and MRV could be improved even if each ton of rock is less efficient. There are many more feasible locations and fewer regulatory and social barriers. Frontier's ultimate goal is to remove a trillion tons of CO2, which requires rock mass equivalent to Mount Everest.
"How cheap can weathering a giant pile of rocks be?" becomes the question.
Balancing the Costs and Challenges
Basic rocks available in the trillions of tons tend to have slow reaction rates with atmospheric CO2. It is a challenge to add reactants and remove products efficiently enough for reactions to proceed at their kinetic limits. Rates can slow by orders of magnitude if ion diffusion becomes the limiting factor because diffusion time increases by the square of the distance.
These issues compound because rock is not soluble in air. The reaction happens in a water intermediary. But, neither CO2 nor most rocks are very soluble in water. And there is hardly any CO2 in the air at 400 parts per million, anyway. Inexpensive weathering becomes a mass transport problem as much as a kinetic one.
Reaction Rates and Rock Selection
There are three factors crucial to rock selection:
-
Weathering Rate
-
CO2 Sequestration Amount per Unit Rock
-
Rock Availability and Quality
Determining the weathering rate is quite challenging. The literature has rates for Olivine dissolution that vary from 10^-8 to 10^-13 mol/m2-s at ambient conditions, a 100,000x difference (here, here, here, here, and here). The fastest rates are when the rocks are in acidic solutions, which isn't practical for weathering rock in atmospheric conditions because acidic solutions are poor at absorbing CO2, and acid costs money. The dissolution rates for neutral to alkaline conditions range from 10^-10 to 10^-13 mol/m2-s. Higher pH, high dissolved silica, carbonate precipitation, poor mixing, lack of hydration, and colder temperatures can all lead to several orders of magnitude slower rates and push the rate towards the 10^-13 mol/m2-s type numbers. The reaction rate of many other silicates, like feldspars, tends to be within an order of magnitude of olivine. Rocks with more calcium or magnesium not in silicate structures tend to be faster.
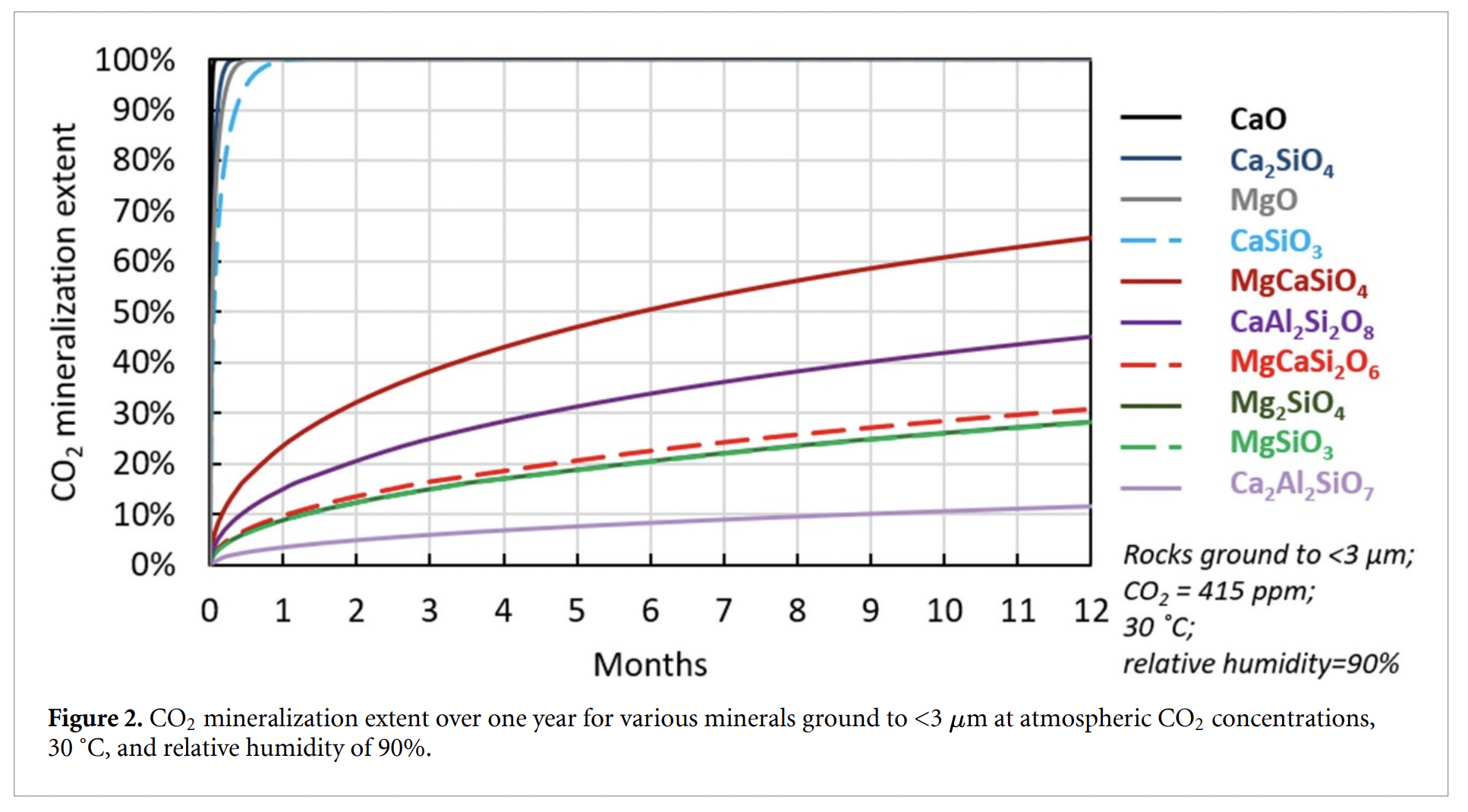
These rates are slower than the "kinetic limit" at these conditions but the particle size is probably smaller than practical. Also, <3 micrometers means the median is less than one micrometer. Source
The capture potential (tons CO2/ton rock) for silicates outside of olivine is usually lower.
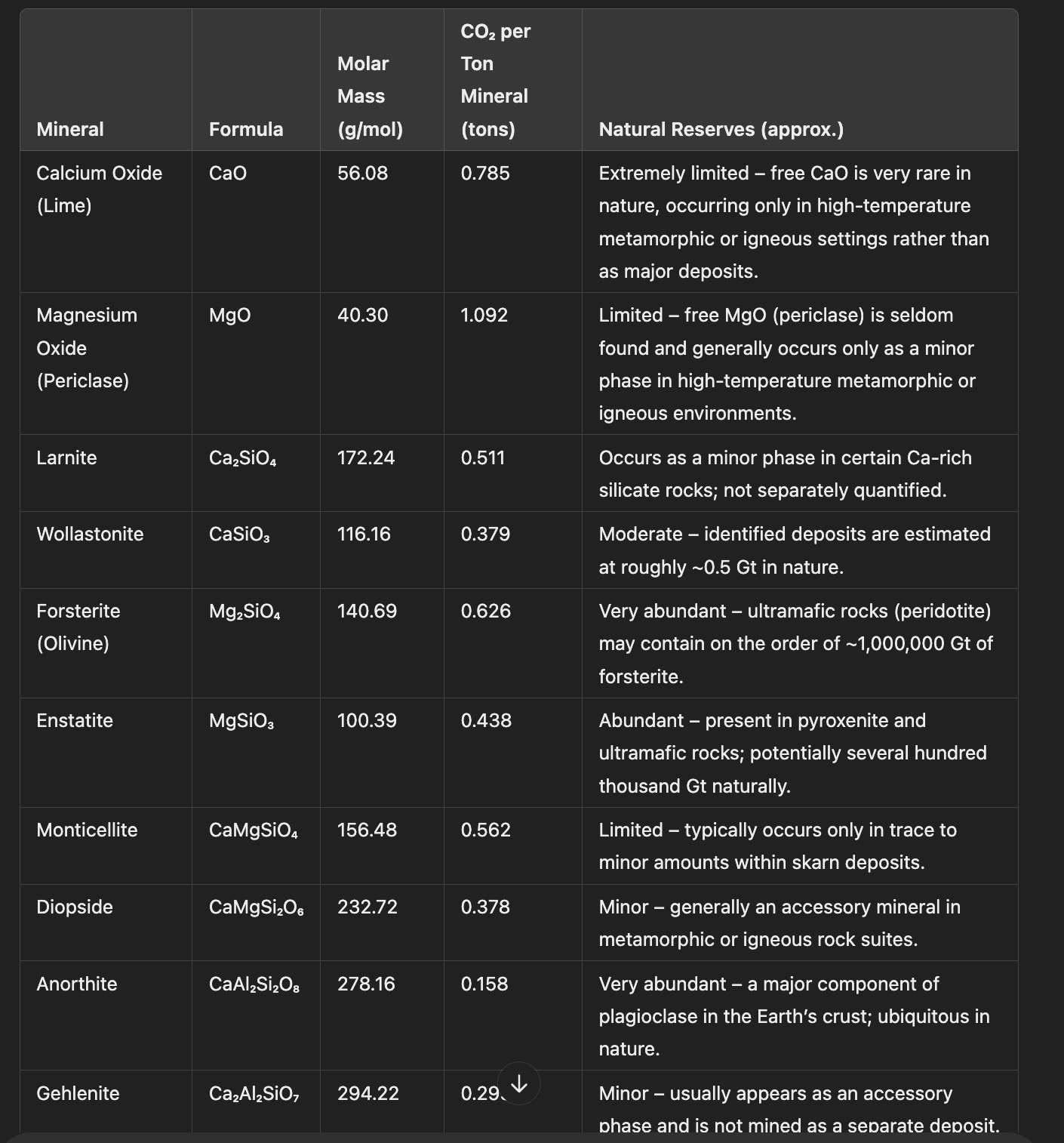
Compiled by my research assistant, ChatGPT
Another important consideration is the rock quality and reserves. The silicate rocks have near-infinite reserves, but many olivines might be in basalts (<0.2 ton CO2/ton rock). Faster-reacting rocks are rare because they are too volatile to last on geological time scales.
The tradeoffs between reaction rate, capture potential, and reserves are why olivine minerals in dunites or peridotites are one of the most common targets for enhanced weathering.
Rock Preparation
Increasing surface area is the simplest way to speed up the reactions, and grinding the rock into small particles is the preferred method.
A note is that sizes are "P80," meaning the size that 80% of the mass is in particles smaller than the given size. The mass-average particle diameter will be smaller, and most particles will be <30% of the size of the P80.
There are several breakpoints:
- Crushed rock down to a few millimeters costs $2-$3 per raw ton of rock.
- Course grinding down to 30 microns with ball mills costs $7-$11 per raw ton of rock.
- Fine grinding to ~10 microns with a stirred mill or similar technology is $12-$15/raw ton.
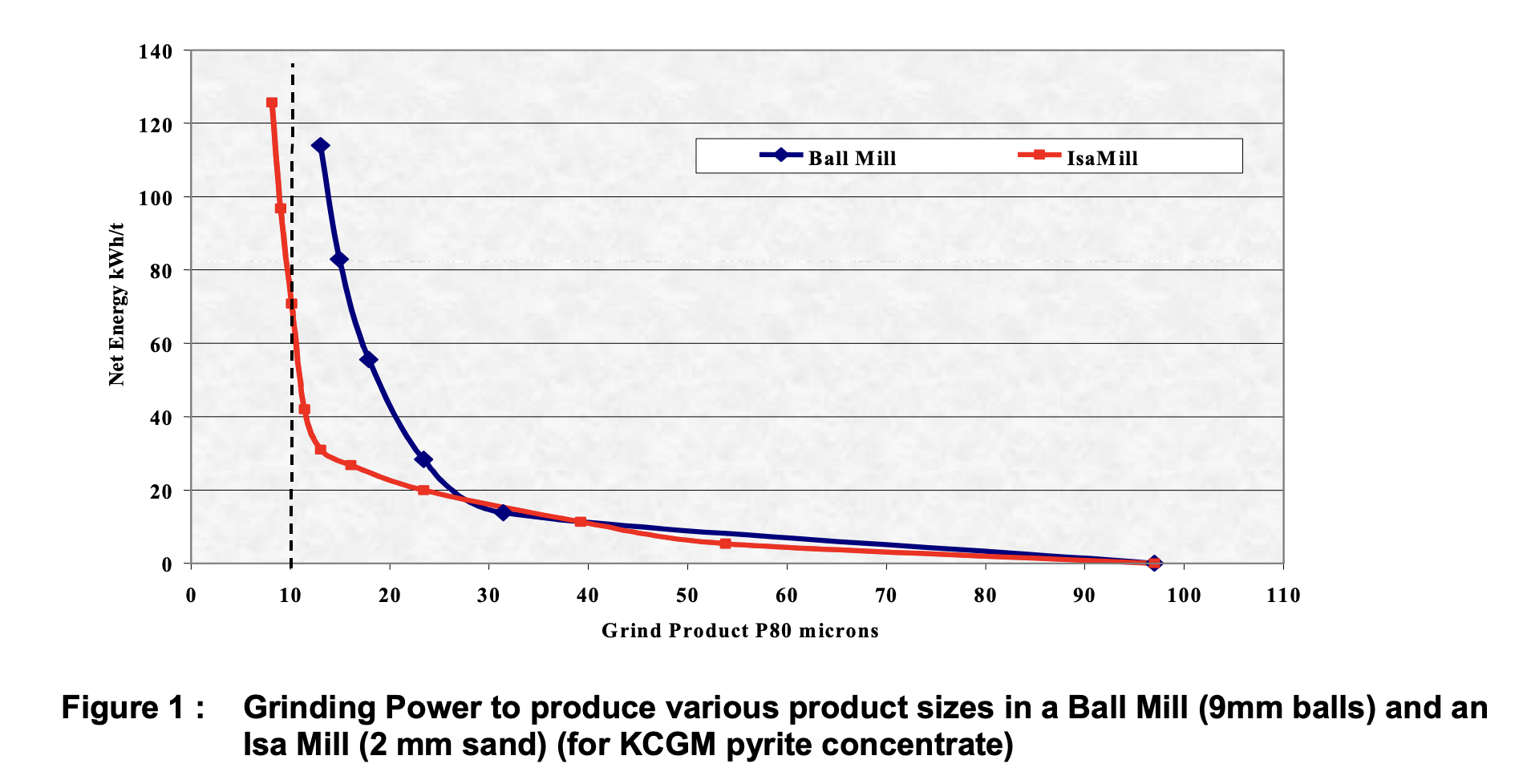
There is a "knee" where further size reduction becomes impractical from an energy, media, and capital efficiency perspective Source
The reaction time at crushed rock scale probably takes thousands of years unless the rock is extremely porous (in which case it is likely already weathered significantly).
Course ground rock would take decades to weather at rates near the kinetic limit.
And fine ground rocks could react in a few years under the right circumstances.
Fine grinding up to the cost "knee" is the obvious choice in most scenarios because it speeds up the reaction 3x while only costing 15%-20% more, reducing working capital requirements.
Air
These processes require extreme air volumes because carbon dioxide's concentration in the atmosphere is so low. Even worse, taking the concentration down to zero is unrealistic because there needs to be a concentration gradient for the reaction to proceed. Cutting the concentration to 200 ppm is probably a reasonable assumption. The process requires roughly 2-3 million cubic meters of air per ton of CO2 (a few cubic kilometers).
Pressure drop must be minimal to prevent energy use and fan horsepower from skyrocketing, which we can estimate using the Ergon Equation.
There is a limit to the distance the air can flow through the rock bed before pressure drop is unrealistic, probably 25-100 meters, depending on rock characteristics. Velocity must also be kept to a minimum, which multi-year reaction times help with. Small particle diameters add tortuosity and increase pressure drop. Any sizes below 25 millimeters become very difficult.
Water
Water is a solvent for carbon dioxide and rock minerals. It also plays a role in the reaction by hydrating cations. Unfortunately, its carrying capacity for gas and rocks is small. Only ~1 mg per liter of CO2 can dissolve in water at atmospheric concentration. Thick layers of water can be a barrier for CO2 to reach the rock since diffusion is so slow. Ca2+ and Mg2+ cation concentrations are typically less than 100 mg per liter. But there must be some water for the reaction to proceed.
An ideal scenario might be a thin, moving film of water flowing across the rock surfaces. Air moving around the rocks can constantly diffuse CO2 into the water. The small distance does not hinder mass transfer. The water can take away products like bicarbonate that slow the dissolution of the rock.
Water rates can be thousands to tens of thousands of meters cubed per ton of CO2 at the extreme where almost all carbon is in solution as bicarbonates and carbonates instead of precipitating in situ. The pumping costs alone can be significant. The maximum water use is only feasible when pumping at very low pressure (a few meters of head). Smaller amounts of water are still helpful in moving products and reactants.
Mineral Precipitation
Carbonates have to precipitate for this style of weathering to be effective.
Most precipitation will happen on the rock surface in the absence of high water flows. The carbonates can form a passivating layer that significantly slows reaction rates. Excess water flow can speed up the weathering reaction rate for this reason.
Precipitation is a surface area-limited process. It can happen away from the weathered rock, but there must be "seed crystals" and surfaces for the concentration of the cations and carbonate anions to approach equilibrium. It helps if the surfaces are the same mineral. Spontaneous precipitation is difficult and requires extensive supersaturation.
Combining Requirements
The analysis reveals the constraints and dilemmas:
-
Total rock surface area is critical, with grinding being the most conventional way to achieve that.
-
The air volumetric flow rate is pretty extreme. The low pressure drop requirement conflicts with using micron-diameter rock particles.
-
Water is helpful, but its use must account for total pumping costs.
-
The formation and precipitation of carbonates are necessary if the carbon is not going into the ocean and can form passivation layers that slow weathering. Water can help this, but again, cost is a barrier.
-
The driving force for the reactions is very weak. Typically, industrial reactions happen quickly with high concentrations of reactants in favorable conditions. The low concentration of CO2 in the atmosphere and the extreme cost constraints eliminate more aggressive options. Most rocks that can weather very quickly are already weathered! These processes at scale must operate on the edge of possibility.
The Initial Concept
The key design issue is resolving the conflict between small particle size and airflow. A previously proposed concept is blowing humid air down a 1-kilometer-long greenhouse with trays full of ground rock. The trays are thin enough that CO2 can diffuse through the powder from the moving air. Alternating layers of boulders and powder could facilitate humid airflow to absorb CO2 in rock piles, similar to the greenhouse.
The particles must be less than 10 microns to have reaction times measured in years instead of centuries.
Alternating big rocks and powder presents some logistical and practical challenges, but there is a better way. One paper proposes a mining method called heap leaching, where water is trickled down piles that can have millions of tons of rock. Miners press finely ground particles into porous and permeable pellets up to 25 mm in diameter in these piles, solving the air pressure drop issues.
The water sprinkling in heap leach piles would also speed up the reaction much more than simple air humidification. Nina and Jenny from Orca Sciences ran experiments on various water and air contact methods. They confirmed that a thin film of water on particles sped up the reaction by orders of magnitude compared to humid air or excess water.
Measurement, reporting, and verification happen by measuring the CO2 concentration of air coming in and out of the pile if the water is closed loop. CO2 is infrared active, so online measurement is very affordable. An MRV setup like this would be much better than the methods for other forms of enhanced weathering, like ocean disposal or spreading rock dust on fields.
The concept ends up being relatively low-risk and scalable.
Sourcing and Cost Estimates
Note: Cost and engineering calculations are linked in this spreadsheet.
Setting the goal of billions or trillions of tons of carbon removal narrows down the potential sites and rock available.
Basalt, which might sequester 0.15 tons of CO2 per ton rock, is ubiquitous and often has high-quality reserves. Using basalt is ~$110/ton CO2 without discounting ($1/ton adds ~$0.01/gallon to gasoline). In some ways, this is great, as it is cheaper than other solutions available. On the other hand, $110/ton limits the market, and nearly all the cost is upfront. Considering the cost of capital increases the price several times.
Rocks like peridotite, mostly made up of olivine, are common in Earth's mantle and can remove more CO2 per ton of rock. The Earth has enough to sequester hundreds of millions of times the CO2 in the atmosphere in the mantle. But, these mantle rocks are only easily accessible in large quantities in a few places on Earth where geological processes have thrust the mantle closer to the surface. Oman, especially, has enough theoretical reserves to remove all the CO2 in the atmosphere several times over. Again, the issue becomes how large individual pockets are and how much overburden there is to remove.
The rock formations in Oman are well-studied and tend to have two layers. A top highly fractured layer is near equilibrium with the atmosphere. A less disturbed, low permeability peridotite layer below is mostly unreacted. It seems likely that accessibility varies, but 200 meters might be a conservative estimate for overburden. The overburden adds $2-$15/ton CO2 captured if the scale is in the tens of millions of tons.
The range of estimates for peridotite are $35-$85/ton CO2 removed (undiscounted). The variation comes from rock purity, electricity cost, and overburden removal.
Overall, achieving an undiscounted cost of CO2 removal that is less than $100/ton at the billions or even trillions of tons scale seems doable. And while the market might not support that scale of effort, there is a profound impact on policy. Most environmental subsidies cost more than $100/ton of CO2. For example, assume an existing natural gas power plant costs $40/MWh to run and emits 0.4 tons CO2/MWh. Replacement generation that costs $100/MWh would have an abatement cost of $150/ton of CO2. Targeted subsidies for specific technologies look even worse compared to blanket carbon taxes/removals than they did before. $1 billion would buy a fully-scaled first site to prove that globally relevant weathering amounts are viable. Field studies to verify a location and rock reactivity might cost 1/100th of that.
Here's to a future where every Omani is a millionaire from peridotite excise taxes.
Pushing for Transformational Costs
Naturally, the next question is if there is a way to work around the constraints to drive even lower costs. Sometimes, it can be helpful to separate steps if they have conflicting requirements. Each process can have optimized conditions. Testing and evaluation can also be simpler by doing one step at a time.
There might be several possible ways to do this in Oman or elsewhere.
Magnesium and One Pond
The reaction rates impact the final cost through the cost of capital. One possible way to reduce these costs is to improve rock dissolution by submerging pellets in a pond and mixing air into the water.
Purely liquid phase leaching with fluid circulation can disperse the chemical reaction products and reactants. The rock dissolution rate can be 5x-50x faster, getting much closer to the 10^-10 mol/m2-s rates, shrinking time by 10-100x, or allowing a 10x-100x increase in particle diameter.
The process goes as follows:
-
The pH of the solution rises as the rock dissolves, and the higher pH water travels through an air contactor to capture CO2.
-
A spray column air contactor is simple and can provide low pressure drop for air and water, keeping pumping costs reasonable.
-
The CO2 concentration at the inlet and outlet can verify the carbon removal.
-
Mixing air and water lowers the pH and creates more carbonate and bicarbonate ions.
-
These anions react with cations from leaching, leading to precipitation.
-
The leaching and precipitation need to happen near each other in magnesium-dominated systems because magnesium carbonates have high solubility compared to many carbonates and are hard to precipitate without the continuous addition of magnesium ions.
-
Luckily, precipitation heavily favors seed crystals of the same species. A small amount of magnesium carbonate "seed" might limit how much passivation happens to the leaching rock.
It might also be possible to have the pond at the groundwater level so there is no need for pond liners. The ponds will likely be very rectangular, with many pumps spaced apart to limit the fluid flow length and pressure drop. There must be rapid circulation to keep products from building and reactants from becoming scarce.
Keeping electricity costs low is critical for pumping water and air. Removing one ton of CO2 might require pumping as many as 25,000 cubic meters of water.
Makeup water can come directly from the ocean or indirectly by humidifying/saturating the inlet air. Using seawater directly would be cheaper initially but could negatively impact the dissolution and precipitation process.
One feature of a pond setup is that it is easier to test and replicate than the heap leach piles. Each process is isolated and homogenous instead of all the processes happening in a thin film of water that can have huge variances depending on small changes in fluid flow rates or rock geometry. A beaker will be representative of a large pond as long as the pond has adequate circulation. The overhead and R+D needed to build confidence in the process should be lower.
Cheap electricity can reduce discounted costs by accelerating reaction rates via pumps and fans.
The total cost could fall by over fifty percent compared to heap leach pads and be under $100/ton.
Calcium and Leaving the Rock Where It Is
A more speculative idea would be circulating water through the rock instead of mining it. Some efforts remove carbon dioxide by mixing it with water and pumping it underground to react with the rock and form carbonates. An issue with this method is that it requires high partial-pressure carbon dioxide to be effective and keep pumping costs reasonable. Another problem is that the precipitating minerals clog up fractures and porosity, limiting throughput.
A variation on the architecture would be to separate the rock dissolution and precipitation to prevent clogging. An interesting feature of deep hyperalkaline groundwater in Oman is that calcium is the dominant cation, and very little magnesium is present. Calcium hydroxide has much higher solubility than magnesium hydroxide, so the Mg(OH)2 precipitates as pH increases, leaving calcium ions. On the precipitation side, calcium carbonate precipitates at a much lower concentration than magnesium carbonates. The net result is that the carbon-carrying capacity of these deep reservoirs is much higher than magnesium-dominant waters because the maximum calcium concentration during dissolution is higher, and the minimum after precipitation is lower.
A pair of wells could circulate water deep underground and solution mine the calcium from the rocks. The bottom hole temperature could be as high as 150C, increasing the rate by several orders of magnitude. There would still be an air contactor at the surface and a small precipitation pond to remove calcite. It may be possible to avoid pumping costs using natural circulation because of the top and bottom temperature differences. The hot water might also help drive airflow at the surface.
Viability depends on permeability in the system. The volumes with the most unreacted rock should be the least permeable. There must be an increase in permeability. That might come from fracking, but could also be something more mining-related, like explosives.
The geometry would differ from enhanced geothermal wells because the water volumes are much larger (and a cubic meter of rock produces more value capturing carbon). Wells might only be a few tens of meters apart with thousands of meters of vertical connection. Permeability would still ideally be approaching that of a gravel bar. Pumping pressure could be 20x-50x higher than the pond configuration because of calcium-based fluid’s better carbon carrying capacity and help from density-driven convection. The hole diameter must be as large as is possible with typical drilling rigs to facilitate water flow.
The system is more challenging to test but could be scalable in more locations because it is the least sensitive to rock quality. Calcium-rich formations in the US, like those of Ca-feldspar, are plentiful (though there is some concern about secondary clay formation sapping calcium ions). They could benefit from US supply chains and financing while being more accessible than Oman.
The costs for solution mining calcium might be <$20/ton CO2 under the most optimistic scenarios with a fraction of the surface disturbance.
Conclusion
Industrial-style, land-based weathering can solve many scaling, cost, and verification barriers that plague other varieties like ocean disposal or field application. These three methods have a chance of being cheaper than the majority of subsidized decarbonization technologies. Testing these methods could cost as little as a few million dollars, and scale is practically infinite if they work.
A Review of Massively Scalable Enhanced Rock Weathering
2025 April 22 Twitter Substack See all postsHow cheap can it pull CO2 from the air? Thanks to Nina, Jenny, Fridrik, Nan, Dan, Judy, and Frauke for their help and teaching on this subject. Any mistakes are my own.
Prompt
Enhanced rock weathering is a method to remove carbon dioxide from the atmosphere and store it permanently. Some of its best features are simplicity, permanence, and scale. The mechanism is that carbon dioxide in the air reacts with rock particles to form stable carbonates. There are many times more rocks than we need to remove industrial-era emissions.
"Why aren't we grinding up mountains yet?" is a question I've been curious about. Folks from Stripe Frontier and Orca Sciences have taught me a lot about the topic.
The promise is still there, but the road is more challenging than at first glance. The difficulty increases after considering rock quality, reaction kinetics, logistics, ocean impacts, mining operations, and MRV (Measurement, Reporting, and Verification).
Intro
We have more carbon dioxide in the atmosphere than we'd like, and it is an acid gas. Acid gases form acidic solutions when they dissolve in water. The magic of enhanced rock weathering is finding basic rocks that can react with and neutralize CO2 into bicarbonate or precipitated carbonates. Below are the reactions for olivine, which is one of the most commonly proposed rock types:
Mg2SiO4 + 4CO2 + 2H2O => 2Mg2+ + 4HCO3- + SiO2
Each ton of olivine removes 1.25 tons of CO2 in the aqueous reaction. This reaction is only relevant for dumping the rocks in the ocean because smaller reservoirs can't maintain that much bicarbonate.
There is also a route that forms carbonates:
Mg2SiO4 + 2CO2 => 2MgCO3 + SiO2
The reaction is two steps, with the first forming bicarbonate ions and the second reaction producing carbonates:
Mg2+ + 2(HCO3-) => MgCO3 + CO2 + H2O
The carbonate reaction does not store as much CO2 as the bicarbonate phase.
Overall, the CO2/bicarbonate/carbonate buffer system is integral to how rock weathering proceeds.
Evaluating Previous Cost Claims
Part of the hype around rock weathering is claims that it could be done for $10/ton of CO2 removed at scale. A cost like this would make a gallon of fuel carbon neutral for only ~$0.10.
Diving further into the costs suggests that the conditions where this might be true are strict:
Tens of millions of tons per year scale.
Extremely large, contiguous, and high purity reserves with minimal overburden.
Inexpensive electricity (<$20/MWh).
Zero cost of capital.
No verification, testing, or pollution concerns.
Ocean disposal to get the bicarbonate bonus.
A mine located less than 5 kilometers from the ocean.
The ocean is the warm water variety to speed reaction rates.
Acceptance of long time horizons or new grinding technology.
It isn't clear whether these conditions are available in any location on Earth. High-quality reserves tend to be in smaller veins within larger deposits. Existing olivine mines are small and far from the ocean or in cold places. Pollution is a concern because deposits usually contain small amounts of metals like nickel that can cause localized issues in the ocean. The material might not fully react before settling on the bottom, and measuring this is challenging.
Quotes for high-purity olivine from existing mines are over $100/ton because of their sub-scale nature and the mining difficulty. One of the main mines in the US, Twin Sisters in Washington, isn't even accessible year-round.
$10/ton does not seem realistic.
Land-Based Weathering
Nan, Dan, and Judy at Frontier suggested that land-based weathering might be under-explored because transportation, pollution, land use, and MRV could be improved even if each ton of rock is less efficient. There are many more feasible locations and fewer regulatory and social barriers. Frontier's ultimate goal is to remove a trillion tons of CO2, which requires rock mass equivalent to Mount Everest.
"How cheap can weathering a giant pile of rocks be?" becomes the question.
Balancing the Costs and Challenges
Basic rocks available in the trillions of tons tend to have slow reaction rates with atmospheric CO2. It is a challenge to add reactants and remove products efficiently enough for reactions to proceed at their kinetic limits. Rates can slow by orders of magnitude if ion diffusion becomes the limiting factor because diffusion time increases by the square of the distance.
These issues compound because rock is not soluble in air. The reaction happens in a water intermediary. But, neither CO2 nor most rocks are very soluble in water. And there is hardly any CO2 in the air at 400 parts per million, anyway. Inexpensive weathering becomes a mass transport problem as much as a kinetic one.
Reaction Rates and Rock Selection
There are three factors crucial to rock selection:
Weathering Rate
CO2 Sequestration Amount per Unit Rock
Rock Availability and Quality
Determining the weathering rate is quite challenging. The literature has rates for Olivine dissolution that vary from 10^-8 to 10^-13 mol/m2-s at ambient conditions, a 100,000x difference (here, here, here, here, and here). The fastest rates are when the rocks are in acidic solutions, which isn't practical for weathering rock in atmospheric conditions because acidic solutions are poor at absorbing CO2, and acid costs money. The dissolution rates for neutral to alkaline conditions range from 10^-10 to 10^-13 mol/m2-s. Higher pH, high dissolved silica, carbonate precipitation, poor mixing, lack of hydration, and colder temperatures can all lead to several orders of magnitude slower rates and push the rate towards the 10^-13 mol/m2-s type numbers. The reaction rate of many other silicates, like feldspars, tends to be within an order of magnitude of olivine. Rocks with more calcium or magnesium not in silicate structures tend to be faster.
These rates are slower than the "kinetic limit" at these conditions but the particle size is probably smaller than practical. Also, <3 micrometers means the median is less than one micrometer. Source
The capture potential (tons CO2/ton rock) for silicates outside of olivine is usually lower.
Compiled by my research assistant, ChatGPT
Another important consideration is the rock quality and reserves. The silicate rocks have near-infinite reserves, but many olivines might be in basalts (<0.2 ton CO2/ton rock). Faster-reacting rocks are rare because they are too volatile to last on geological time scales.
The tradeoffs between reaction rate, capture potential, and reserves are why olivine minerals in dunites or peridotites are one of the most common targets for enhanced weathering.
Rock Preparation
Increasing surface area is the simplest way to speed up the reactions, and grinding the rock into small particles is the preferred method.
A note is that sizes are "P80," meaning the size that 80% of the mass is in particles smaller than the given size. The mass-average particle diameter will be smaller, and most particles will be <30% of the size of the P80.
There are several breakpoints:
There is a "knee" where further size reduction becomes impractical from an energy, media, and capital efficiency perspective Source
The reaction time at crushed rock scale probably takes thousands of years unless the rock is extremely porous (in which case it is likely already weathered significantly).
Course ground rock would take decades to weather at rates near the kinetic limit.
And fine ground rocks could react in a few years under the right circumstances.
Fine grinding up to the cost "knee" is the obvious choice in most scenarios because it speeds up the reaction 3x while only costing 15%-20% more, reducing working capital requirements.
Air
These processes require extreme air volumes because carbon dioxide's concentration in the atmosphere is so low. Even worse, taking the concentration down to zero is unrealistic because there needs to be a concentration gradient for the reaction to proceed. Cutting the concentration to 200 ppm is probably a reasonable assumption. The process requires roughly 2-3 million cubic meters of air per ton of CO2 (a few cubic kilometers).
Pressure drop must be minimal to prevent energy use and fan horsepower from skyrocketing, which we can estimate using the Ergon Equation.
There is a limit to the distance the air can flow through the rock bed before pressure drop is unrealistic, probably 25-100 meters, depending on rock characteristics. Velocity must also be kept to a minimum, which multi-year reaction times help with. Small particle diameters add tortuosity and increase pressure drop. Any sizes below 25 millimeters become very difficult.
Water
Water is a solvent for carbon dioxide and rock minerals. It also plays a role in the reaction by hydrating cations. Unfortunately, its carrying capacity for gas and rocks is small. Only ~1 mg per liter of CO2 can dissolve in water at atmospheric concentration. Thick layers of water can be a barrier for CO2 to reach the rock since diffusion is so slow. Ca2+ and Mg2+ cation concentrations are typically less than 100 mg per liter. But there must be some water for the reaction to proceed.
An ideal scenario might be a thin, moving film of water flowing across the rock surfaces. Air moving around the rocks can constantly diffuse CO2 into the water. The small distance does not hinder mass transfer. The water can take away products like bicarbonate that slow the dissolution of the rock.
Water rates can be thousands to tens of thousands of meters cubed per ton of CO2 at the extreme where almost all carbon is in solution as bicarbonates and carbonates instead of precipitating in situ. The pumping costs alone can be significant. The maximum water use is only feasible when pumping at very low pressure (a few meters of head). Smaller amounts of water are still helpful in moving products and reactants.
Mineral Precipitation
Carbonates have to precipitate for this style of weathering to be effective.
Most precipitation will happen on the rock surface in the absence of high water flows. The carbonates can form a passivating layer that significantly slows reaction rates. Excess water flow can speed up the weathering reaction rate for this reason.
Precipitation is a surface area-limited process. It can happen away from the weathered rock, but there must be "seed crystals" and surfaces for the concentration of the cations and carbonate anions to approach equilibrium. It helps if the surfaces are the same mineral. Spontaneous precipitation is difficult and requires extensive supersaturation.
Combining Requirements
The analysis reveals the constraints and dilemmas:
Total rock surface area is critical, with grinding being the most conventional way to achieve that.
The air volumetric flow rate is pretty extreme. The low pressure drop requirement conflicts with using micron-diameter rock particles.
Water is helpful, but its use must account for total pumping costs.
The formation and precipitation of carbonates are necessary if the carbon is not going into the ocean and can form passivation layers that slow weathering. Water can help this, but again, cost is a barrier.
The driving force for the reactions is very weak. Typically, industrial reactions happen quickly with high concentrations of reactants in favorable conditions. The low concentration of CO2 in the atmosphere and the extreme cost constraints eliminate more aggressive options. Most rocks that can weather very quickly are already weathered! These processes at scale must operate on the edge of possibility.
The Initial Concept
The key design issue is resolving the conflict between small particle size and airflow. A previously proposed concept is blowing humid air down a 1-kilometer-long greenhouse with trays full of ground rock. The trays are thin enough that CO2 can diffuse through the powder from the moving air. Alternating layers of boulders and powder could facilitate humid airflow to absorb CO2 in rock piles, similar to the greenhouse.
The particles must be less than 10 microns to have reaction times measured in years instead of centuries.
Alternating big rocks and powder presents some logistical and practical challenges, but there is a better way. One paper proposes a mining method called heap leaching, where water is trickled down piles that can have millions of tons of rock. Miners press finely ground particles into porous and permeable pellets up to 25 mm in diameter in these piles, solving the air pressure drop issues.
The water sprinkling in heap leach piles would also speed up the reaction much more than simple air humidification. Nina and Jenny from Orca Sciences ran experiments on various water and air contact methods. They confirmed that a thin film of water on particles sped up the reaction by orders of magnitude compared to humid air or excess water.
Measurement, reporting, and verification happen by measuring the CO2 concentration of air coming in and out of the pile if the water is closed loop. CO2 is infrared active, so online measurement is very affordable. An MRV setup like this would be much better than the methods for other forms of enhanced weathering, like ocean disposal or spreading rock dust on fields.
The concept ends up being relatively low-risk and scalable.
Sourcing and Cost Estimates
Note: Cost and engineering calculations are linked in this spreadsheet.
Setting the goal of billions or trillions of tons of carbon removal narrows down the potential sites and rock available.
Basalt, which might sequester 0.15 tons of CO2 per ton rock, is ubiquitous and often has high-quality reserves. Using basalt is ~$110/ton CO2 without discounting ($1/ton adds ~$0.01/gallon to gasoline). In some ways, this is great, as it is cheaper than other solutions available. On the other hand, $110/ton limits the market, and nearly all the cost is upfront. Considering the cost of capital increases the price several times.
Rocks like peridotite, mostly made up of olivine, are common in Earth's mantle and can remove more CO2 per ton of rock. The Earth has enough to sequester hundreds of millions of times the CO2 in the atmosphere in the mantle. But, these mantle rocks are only easily accessible in large quantities in a few places on Earth where geological processes have thrust the mantle closer to the surface. Oman, especially, has enough theoretical reserves to remove all the CO2 in the atmosphere several times over. Again, the issue becomes how large individual pockets are and how much overburden there is to remove.
The rock formations in Oman are well-studied and tend to have two layers. A top highly fractured layer is near equilibrium with the atmosphere. A less disturbed, low permeability peridotite layer below is mostly unreacted. It seems likely that accessibility varies, but 200 meters might be a conservative estimate for overburden. The overburden adds $2-$15/ton CO2 captured if the scale is in the tens of millions of tons.
The range of estimates for peridotite are $35-$85/ton CO2 removed (undiscounted). The variation comes from rock purity, electricity cost, and overburden removal.
Overall, achieving an undiscounted cost of CO2 removal that is less than $100/ton at the billions or even trillions of tons scale seems doable. And while the market might not support that scale of effort, there is a profound impact on policy. Most environmental subsidies cost more than $100/ton of CO2. For example, assume an existing natural gas power plant costs $40/MWh to run and emits 0.4 tons CO2/MWh. Replacement generation that costs $100/MWh would have an abatement cost of $150/ton of CO2. Targeted subsidies for specific technologies look even worse compared to blanket carbon taxes/removals than they did before. $1 billion would buy a fully-scaled first site to prove that globally relevant weathering amounts are viable. Field studies to verify a location and rock reactivity might cost 1/100th of that.
Here's to a future where every Omani is a millionaire from peridotite excise taxes.
Pushing for Transformational Costs
Naturally, the next question is if there is a way to work around the constraints to drive even lower costs. Sometimes, it can be helpful to separate steps if they have conflicting requirements. Each process can have optimized conditions. Testing and evaluation can also be simpler by doing one step at a time.
There might be several possible ways to do this in Oman or elsewhere.
Magnesium and One Pond
The reaction rates impact the final cost through the cost of capital. One possible way to reduce these costs is to improve rock dissolution by submerging pellets in a pond and mixing air into the water.
Purely liquid phase leaching with fluid circulation can disperse the chemical reaction products and reactants. The rock dissolution rate can be 5x-50x faster, getting much closer to the 10^-10 mol/m2-s rates, shrinking time by 10-100x, or allowing a 10x-100x increase in particle diameter.
The process goes as follows:
The pH of the solution rises as the rock dissolves, and the higher pH water travels through an air contactor to capture CO2.
A spray column air contactor is simple and can provide low pressure drop for air and water, keeping pumping costs reasonable.
The CO2 concentration at the inlet and outlet can verify the carbon removal.
Mixing air and water lowers the pH and creates more carbonate and bicarbonate ions.
These anions react with cations from leaching, leading to precipitation.
The leaching and precipitation need to happen near each other in magnesium-dominated systems because magnesium carbonates have high solubility compared to many carbonates and are hard to precipitate without the continuous addition of magnesium ions.
Luckily, precipitation heavily favors seed crystals of the same species. A small amount of magnesium carbonate "seed" might limit how much passivation happens to the leaching rock.
It might also be possible to have the pond at the groundwater level so there is no need for pond liners. The ponds will likely be very rectangular, with many pumps spaced apart to limit the fluid flow length and pressure drop. There must be rapid circulation to keep products from building and reactants from becoming scarce.
Keeping electricity costs low is critical for pumping water and air. Removing one ton of CO2 might require pumping as many as 25,000 cubic meters of water.
Makeup water can come directly from the ocean or indirectly by humidifying/saturating the inlet air. Using seawater directly would be cheaper initially but could negatively impact the dissolution and precipitation process.
One feature of a pond setup is that it is easier to test and replicate than the heap leach piles. Each process is isolated and homogenous instead of all the processes happening in a thin film of water that can have huge variances depending on small changes in fluid flow rates or rock geometry. A beaker will be representative of a large pond as long as the pond has adequate circulation. The overhead and R+D needed to build confidence in the process should be lower.
Cheap electricity can reduce discounted costs by accelerating reaction rates via pumps and fans.
The total cost could fall by over fifty percent compared to heap leach pads and be under $100/ton.
Calcium and Leaving the Rock Where It Is
A more speculative idea would be circulating water through the rock instead of mining it. Some efforts remove carbon dioxide by mixing it with water and pumping it underground to react with the rock and form carbonates. An issue with this method is that it requires high partial-pressure carbon dioxide to be effective and keep pumping costs reasonable. Another problem is that the precipitating minerals clog up fractures and porosity, limiting throughput.
A variation on the architecture would be to separate the rock dissolution and precipitation to prevent clogging. An interesting feature of deep hyperalkaline groundwater in Oman is that calcium is the dominant cation, and very little magnesium is present. Calcium hydroxide has much higher solubility than magnesium hydroxide, so the Mg(OH)2 precipitates as pH increases, leaving calcium ions. On the precipitation side, calcium carbonate precipitates at a much lower concentration than magnesium carbonates. The net result is that the carbon-carrying capacity of these deep reservoirs is much higher than magnesium-dominant waters because the maximum calcium concentration during dissolution is higher, and the minimum after precipitation is lower.
A pair of wells could circulate water deep underground and solution mine the calcium from the rocks. The bottom hole temperature could be as high as 150C, increasing the rate by several orders of magnitude. There would still be an air contactor at the surface and a small precipitation pond to remove calcite. It may be possible to avoid pumping costs using natural circulation because of the top and bottom temperature differences. The hot water might also help drive airflow at the surface.
Viability depends on permeability in the system. The volumes with the most unreacted rock should be the least permeable. There must be an increase in permeability. That might come from fracking, but could also be something more mining-related, like explosives.
The geometry would differ from enhanced geothermal wells because the water volumes are much larger (and a cubic meter of rock produces more value capturing carbon). Wells might only be a few tens of meters apart with thousands of meters of vertical connection. Permeability would still ideally be approaching that of a gravel bar. Pumping pressure could be 20x-50x higher than the pond configuration because of calcium-based fluid’s better carbon carrying capacity and help from density-driven convection. The hole diameter must be as large as is possible with typical drilling rigs to facilitate water flow.
The system is more challenging to test but could be scalable in more locations because it is the least sensitive to rock quality. Calcium-rich formations in the US, like those of Ca-feldspar, are plentiful (though there is some concern about secondary clay formation sapping calcium ions). They could benefit from US supply chains and financing while being more accessible than Oman.
The costs for solution mining calcium might be <$20/ton CO2 under the most optimistic scenarios with a fraction of the surface disturbance.
Conclusion
Industrial-style, land-based weathering can solve many scaling, cost, and verification barriers that plague other varieties like ocean disposal or field application. These three methods have a chance of being cheaper than the majority of subsidized decarbonization technologies. Testing these methods could cost as little as a few million dollars, and scale is practically infinite if they work.